The advent of direct detectors has led to a resolution revolution in transmission electron microscopy, particularly in the biological sciences [1]. When operated in electron counting mode, monolithic active pixel sensor (MAPS) direct detectors with large pixel areas (e.g. 4096 x 4096 pixels) can achieve detective quantum efficiencies close to the theoretical maximum [2]. This enables high-resolution imaging of a specimen over a wide field of view at relatively low electron beam doses. One example of the impact of electron counting MAPS detectors have had is the steadily increasing number of biological structures that can be resolved to 4 Å or better using single particle cryo-electron microscopy (CryoEM) [3].
Challenges for MAPS Detectors
However, there remain areas for further improvement of MAPS detectors. One of these challenges is to reduce the high financial cost of these detectors, which can sometimes exceed AUD$1,000,000. A second challenge is that very high DQE imaging via electron counting with a MAPS detector is only possible under a relatively narrow range of beam dose conditions. This can impose restrictions for experiments where the optimum imaging conditions for the detector may differ from the optimum imaging conditions for the specimen.
Overcoming MAPS Detector Challenges with Apollo
Apollo from Direct Electron is a 4096 x 4096 pixel MAPS detector with an 8 µm pixel pitch that was designed to simultaneously address both of the challenges described above. Apollo does so using event-based readout of data from its sensor, as opposed to the traditional frame-based readout method used in almost all other electron microscopy detectors. As illustrated in Figure 1, and explained in further detail in a recent article in Microscopy & Analysis magazine [4], an event-based readout is a data-efficient alternative to traditional frame-based readout for applications that involve a sparse signal, as is the case for electron counting. Unlike a frame-based detector, Apollo only reads pixels that register an intensity change due to excitation by an electron, ignoring pixels that contain no signal. This greatly reduces the data bandwidth from the sensor, reducing power consumption, and the cost of the system. Although data is read from the sensor hardware using an event-based method, Apollo still outputs images in standard formats to its computer system. A field programmable gate array (FPGA) inside the camera compiles the detected electron events into 8192 x 8192 pixel super-resolution images, which are transmitted to a computer for storage on disk at a rate of up to 60 images per second.
Advantages of Event-Based MAPS Detectors
The event-based readout from the sensor further allows Apollo to count electrons more rapidly than current state-of-the-art frame-based MAPS detectors. This is evident from published measurements of the incident electron beam dose rate per pixel vs the coincidence loss on different detectors [5-7]. Coincidence loss is a fundamental limiting factor for electron counting detectors and occurs when two or more electrons strike the detector too closely in space and time, resulting in them being miscounted, and signal being lost. At any given coincidence loss level, Apollo is able to count electrons more rapidly than a frame-based detector. At the 5% coincidence loss level, Apollo can operate at beam dose rates of up to 15 electrons per pixel per second (e/pix/s), meaning it is able to count electrons almost twice as rapidly as the nearest frame-based detector, for which the maximum beam dose rate at a 5% level of coincidence loss is only 8 e/pix/s [4].
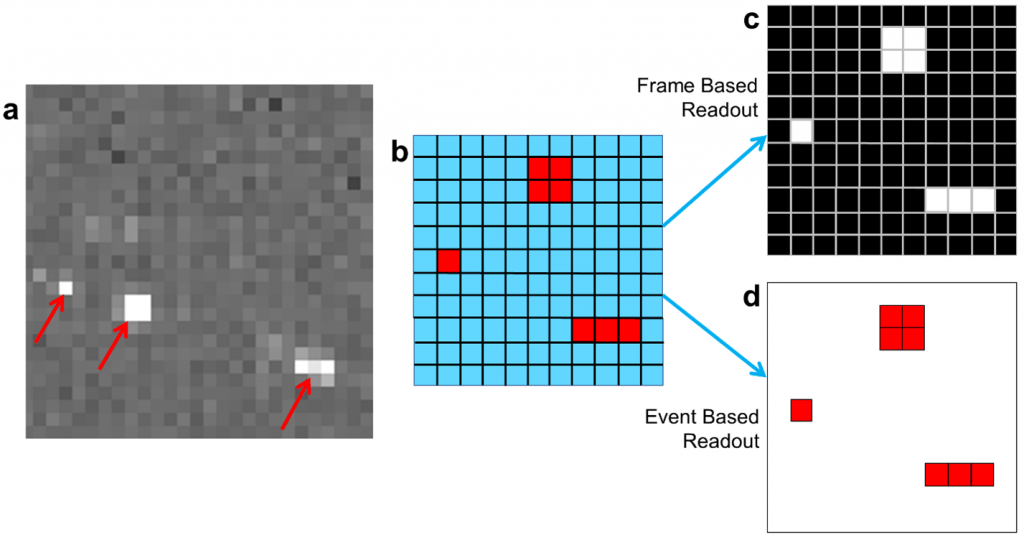
CryoEM Applications
To date, the principal application of the Apollo detector has been single particle CryoEM. Figure 2 shows early results obtained by researchers using the Apollo detector from apoferritin, reconstructed to 1.46 Å [8], recorded on a JEOL CryoARM 300 microscope. A recent publication compared the use of Apollo to state-of-the-art frame-based MAPS detectors for single particle cryo-EM using a Thermo Fisher Scientific Titan Krios microscope [7]. The authors found that for apoferritin, and for a more challenging specimen, adolase, data recorded using Apollo allowed for 3D reconstructions with a higher resolution to be obtained whilst using fewer particles than was possible using the frame-based detectors. Furthermore, the authors found that Apollo’s lower level of coincidence loss allowed greater flexibility in choosing electron beam dose rates for an experiment, facilitating faster experimental throughput [7]. Another recently reported single-particle cryo-EM result from the Apollo is a reconstruction of the tobacco mosaic virus to 1.85 Å resolution, collected by researchers at the University of Glasgow [9]. This result set a record as the highest resolution reconstruction of any specimen obtained by the helical reconstruction technique that has been deposited into the electron microscopy databank at the time of writing this article.
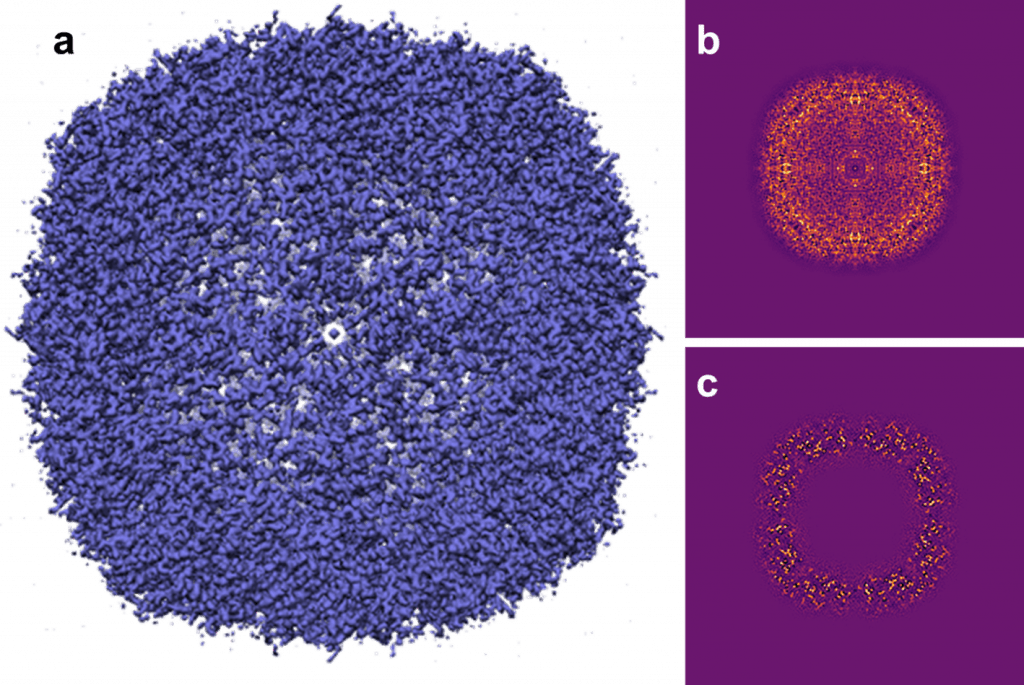
CryoET and MicroED
In addition to single-particle CryoEM, Apollo is actively being explored for use in cryogenic electron tomography, and low dose microcrystal electron diffraction (MicroED). In the case of MicroED, researchers have recently used the Apollo detector to collect a dataset from sodium glutamate, reporting an unprecedentedly high resolution of 0.5 Å in the 3D reconstruction [10].
Materials Science
In materials science, Apollo’s ability to acquire images with very high detective quantum efficiency is valuable for high-resolution TEM imaging of beam sensitive materials specimens. For example, researchers at Ulm University recently used the Apollo detector to image a highly beam-sensitive triazine-based 2D polymer [11]. With a conventional camera, imaging the polymer at lattice resolution was not possible due to radiation damage, but when using the Apollo detector, lattice spacings of 6.7 Å were resolved at a beam dose of only 5 e–/ Å2 [11]. Additional materials science TEM applications likely to benefit from Apollo’s high sensitivity include in situ liquid phase TEM, where the ability to image at a low electron beam dose is often essential for minimising radiation damage to both the specimen, and the liquid environment.
Energy-Filtered TEM
For both biological and materials applications, Apollo is compatible with the CEFID energy filter from CEOS [11,12], and is compatible with microscopes equipped with an Omega Filter, meaning that energy-filtered TEM imaging is another potential application for the detector.
Conclusion
The Apollo direct detection MAPS detector from Direct Electron offers performance levels that exceed those of traditional frame-based readouts. Its event-based readout counts electrons more rapidly and more accurately. Furthermore, by producing a detector that is more affordable, the Apollo makes an excellent option for a detector upgrade for your existing TEM with applications in cryoEM, cryoET, microED and materials science.
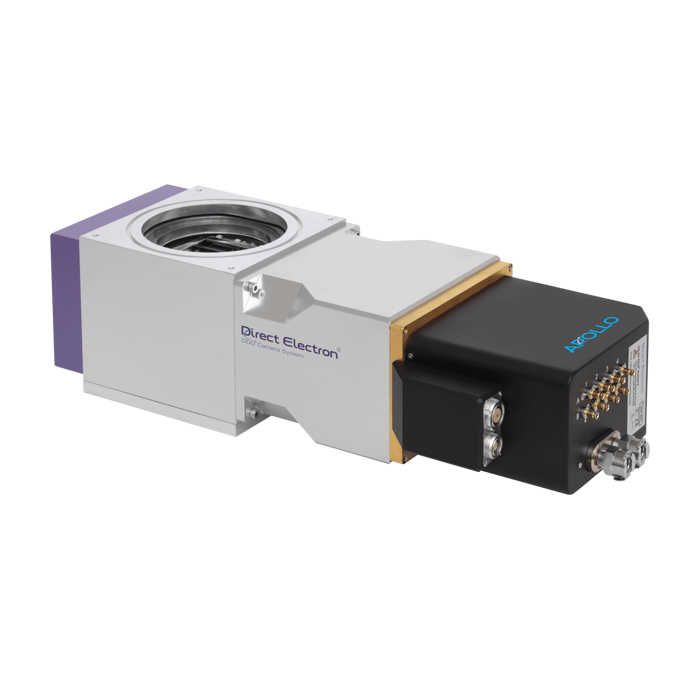
References
[1] Kuhlbrandt W. The resolution revolution. Science 343 1443–44 (2014) https://www.science.org/doi/10.1126/science.1251652
[2] G. McMullan, A.T. Clark, R. Turchetta, A.R. Faruqi, Enhanced imaging in low dose electron microscopy using electron counting, Ultramicroscopy 109 1411–1416 (2009). https://doi.org/10.1016/j.ultramic.2009.07.004
[3] A. Chari & H. Stark, Prospects and Limitations of High-Resolution Single-Particle Cryo-Electron Microscopy Annual Review of Biophysics 52 391-411 (2023) https://doi.org/10.1146/annurev-biophys-111622-091300
[4] B.D.A. Levin, M. Spilman, B. Bammes, Event-based direct detectors and their applications in electron microscopy Microscopy and Analysis 64, 15-17 (2023) https://analyticalscience.wiley.com/do/10.1002/was.000700117
[5] T. Nakane, et al. Single-particle cryo-EM at atomic resolution, Nature 587 152–156 (2020) https://doi.org/10.1038/s41586-020-2829-0.
[6] M. Sun, et al. Practical considerations for using K3 cameras in CDS mode for high-resolution and high-throughput single particle cryo-EM, J. Struct. Biol. 213 107745 (2021). https://doi.org/10.1016/j.jsb.2021.107745
[7] Peng, X. Fu, J.H. Mendez, P.S. Randolph, B.E. Bammes, S.M. Stagg, Characterizing the Resolution and Throughput of the Apollo Direct Electron Detector, J. Struct. Biol. X. 7 100080 (2023). https://doi.org/10.1016/j.yjsbx.2022.100080
[8] B. Bammes, M. Spilman, M. Sakamoto, T. Fukumura, Y. Konyuba, E. Okunishi, Apoferritin structure at 1.46 angstrom resolution by CryoARM300II equipped with Apollo, EMD-33707, https://doi.org/10.6019/EMPIAR-11101
[9] D. Bhella, A.J. Love, J. Streetley, M. Taliansky, K. McGeachy, T. Bukharova, 1.85 angstrom resolution structure of tobacco mosaic virus, imaged on a JEOL CRYOARM 300 and Direct Electron Apollo, EMD-16572, https://doi.org/10.6019/EMPIAR-11404
[10] T. Fukumura, T. Nakane, Y. Konyuba, B. Bammes XRD-00140 (MicroED dataset of sodium glutamate collected with Direct Electron Apollo) XRDa entry DOI: 10.51093/xrd-00140
[11] D. Mücke, M. Linck, G. Guzzinati, H. Müller, B.D.A. Levin, B.E. Bammes, R.G. Brouwer, F. J., H. Qi, U. Kaiser, Effect of self and extrinsic encapsulation on electron resilience of porous 2D polymer nanosheets, Micron, 174, 103525 (2023) https://doi.org/10.1016/j.micron.2023.103525
[12] F. Kahl, V. Gerheim, M. Linck, H. Müller, R. Schillinger, S. Uhlemann, Test and characterization of a new post-column imaging energy filter, in: Adv. Imaging Electron Phys., Elsevier, 35–70 (2019). https://doi.org/10.1016/bs.aiep.2019.08.005